Researchers in Berkeley Lab's Materials Sciences
Division (MSD), working with crystal-growing teams at Cornell University
and Japan's Ritsumeikan University, have learned that the bandgap of the
semiconductor indium nitride is not 2 electron volts (2 eV) as previously
thought, but is close to 0.7 eV.
A technicality? Hardly. The photoelectronic properties of indium, gallium,
and nitrogen alloyed together are well known at higher bandgaps, corresponding
to low indium content. The low bandgap of indium nitride suggests that
by simply varying proportions of indium and gallium, it may be possible
to create rugged, inexpensive devices that can convert the full spectrum
of sunlight to electric current. If so, these could be the most efficient
solar cells ever created.
|
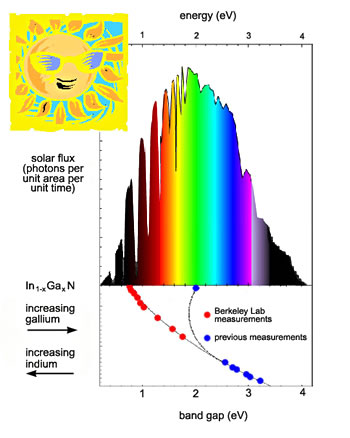 |
|
The indium gallium nitride series
of alloys is photoelectronically active over virtually the entire
range of the solar spectrum. |
|
"It's as if nature designed this material on purpose to match the
solar spectrum," says MSD's Wladek Walukiewicz, who led the collaboration
that made the discovery.
Why bandgaps matter
Bandgaps fundamentally limit the colors a solar cell can convert to electricity.
A semiconductor's bandgap is not a physical space; rather it is the difference
between the energy of the electrons in its filled valence band and the
energy electrons would need to occupy its empty conduction band.
Charge cannot flow in either a completely full or a completely empty
band, but doping a semiconductor provides extra electrons or positively
charged "holes" that can carry a current. Photons with just
the right energy -- the color of light that matches the bandgap -- create
electron-hole pairs and let current flow across the junction between positively
and negatively doped layers.
Photons with less energy than the bandgap slip right through the material.
Photons with too much are absorbed, but since each creates just one electron-hole
pair, the excess energy is wasted as heat.
A one-layer solar cell with a single bandgap can theoretically reach
a maximum of about 30 percent efficiency in converting light to power.
The best efficiency achieved so far, using gallium arsenide with a 1.43
eV bandgap, is about 25 percent. To do better, researchers and manufacturers
stack materials with different bandgaps in so-called multijunction cells.
|
|
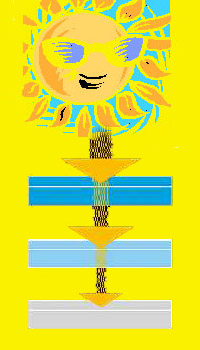 |
 |
|
|
A one-layer solar cell is limited
to 30 percent efficiency in converting light to power, but materials
with different bandgaps can be stacked in multijunction cells. Each
layer responds to a different energy of sunlight. |
|
|
|
In principle, dozens of different layers could be stacked to catch photons
at all energies, for efficiencies better than 70 percent -- but a host
of problems intervenes. If the dimensions of adjacent crystal lattices
differ too much, for example, strain damages the crystals. Other limits
are imposed by opacity, poor heat capacity, and the need in some materials
for thick layers to absorb photons.
Most solar cells are made from silicon. Cheap, amorphous silicon-based
solar cells have efficiencies of less than 10 percent, and the efficiencies
of even the most advanced single-crystal silicon cells are limited to
about 21 percent.
That's because silicon is an "indirect bandgap " semiconductor,
in which creation of an electron-hole pair requires participation of the
crystal lattice vibrations, wasting a lot of an incoming photon's energy.
In direct bandgap semiconductors, however, light of the right energy does
not vibrate the lattice; thus it creates electron-hole pairs more efficiently.
All direct-bandgap semiconductors combine elements from group III of
the periodic table, like aluminum, gallium, or indium, with elements from
group V, like nitrogen, phosphorus, or arsenic. The most efficient multijunction
solar cell yet made -- 30 percent, out of a theoretically possible 50
percent efficiency -- combines just two materials, gallium arsenide and
gallium indium phosphide.
Gallium indium phosphide is a "ternary" compound, in which
two elements from group III are alloyed with one from group V. It was
Berkeley Lab's investigation of a related ternary compound that opened
startling new possibilities for multijunction solar cells. The first clues
came not from studying how semiconductors absorb light to create electrical
power -- but from the reverse.
A nearly perfect solar cell,
part 2
|